
The tomography endstation, based on Fresnel zone plate (FZP) optics, is currently under development. Examples of experiments performed are diffraction and strain mapping of nano-wires (Chayanun et al., 2019 ▸ Hammarberg et al., 2020 ▸ Dzhigaev et al., 2020 ▸ Marçal et al., 2020 ▸) single nano-particle coherent Bragg imaging (Björling et al., 2019 ▸, 2020 b ▸ Dzhigaev et al., 2021 ▸) extreme pressure nano-diffraction (Ji et al., 2020 ▸) ptychographic tomography (Kahnt et al., 2020 ▸) 2D XRF imaging of plant, animal and human cells (Silva Barreto et al., 2020 ▸) nano-diffraction (Nissilä et al., 2021 ▸) and X-ray technology development (Akan et al., 2020 ▸ Chayanun et al., 2020 ▸). The main categories of methods regularly used are CDI in forward and Bragg geometries, nano-diffraction in both geometries, and 2D XRF and X-ray absorption near-edge structure spectroscopy (XANES) imaging. The diffraction endstation, brought into operation in 2017, exploits the intense coherent photon flux, uses Kirkpatrick–Baez (KB) mirrors for focusing to 40–200 nm and is designed to allow for bespoke sample environments and detector configurations. The beamline will have two endstations to allow for diverse experimental requirements regarding sample environment, energy range, resolution or detector configuration. The methods are either based on coherence to achieve spatial resolution, such as in ptychography or coherent diffraction imaging (CDI), or on the focused beam providing spatial resolution, such as in scanning diffraction or X-ray fluorescence (XRF) mapping experiments. NanoMAX is a hard X-ray nanoprobe beamline at MAX IV, designed to accommodate multiple imaging and scattering methods. X-ray fluorescence detectors can handle higher count rates with the latest amplifier and pulse processor technologies (Bordessoule et al., 2019 ▸). Hard X-ray pixel detector performance, such as peak intensity, frame rate and dynamic range, has increased with the most modern photon counting and charge integrating detectors (Ballabriga et al., 2011 ▸ Dinapoli et al., 2013 ▸). On the detector side, the development is also rapid. The gain in coherent flux is utilized in coherent imaging methods with faster acquisition, improved sensitivity, or to achieve smaller and more intense diffraction-limited foci. The development of ultra low-emittance storage rings, first with MAX IV (Tavares et al., 2014 ▸) and soon after ESRF-EBS (Biasci et al., 2014 ▸) and Sirius (Liu et al., 2014 ▸), brings a dramatic increase in coherent flux.
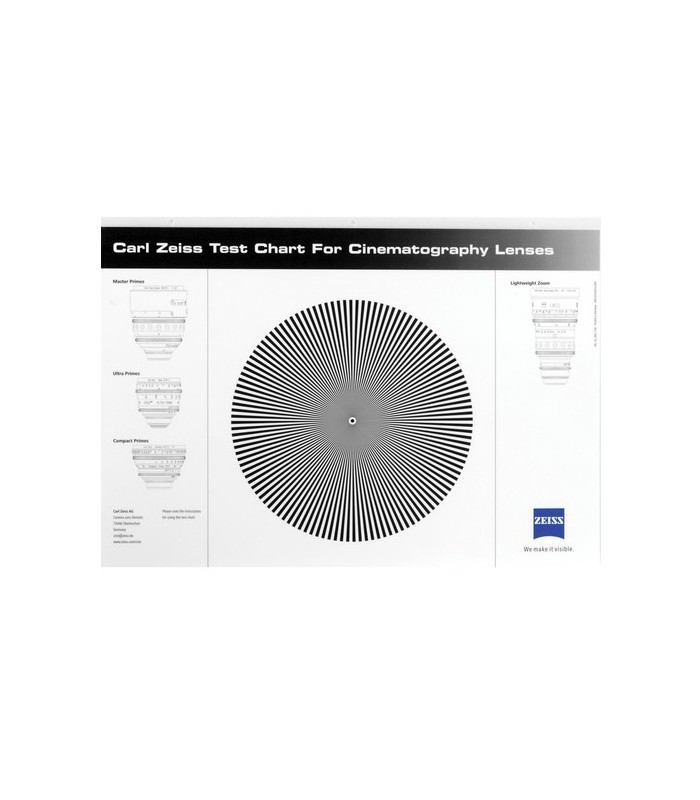
All optics, diffractive, refractive or reflective, have their advantages and disadvantages and no type is best for all energies, focus sizes, working distances and photon flux needs. Focus sizes often reach below 100 nm with examples below 10 nm (Bajt et al., 2018 ▸). Advances in nano-focusing optics fabrication allow focusing to the diffraction limit. A number of technology developments have been important to make SXM attractive to a broader community. Instrument and method development is rapid and most low-emittance synchrotron facilities are today operating or developing one or several hard X-ray nanoprobe beamlines (Leake et al., 2019 ▸ Holler et al., 2018 ▸ Chang et al., 2013 ▸ Chen et al., 2014 ▸ Martínez-Criado et al., 2016 ▸ Nazaretski et al., 2017 ▸ Quinn et al., 2021 ▸ Schropp et al., 2020 ▸ Somogyi et al., 2015 ▸ Tolentino et al., 2017 ▸ Winarski et al., 2012 ▸ de Jonge et al., 2014 ▸). High spatial resolution and large sample penetration depth allow for detailed studies of volume samples, often in their close to natural state.
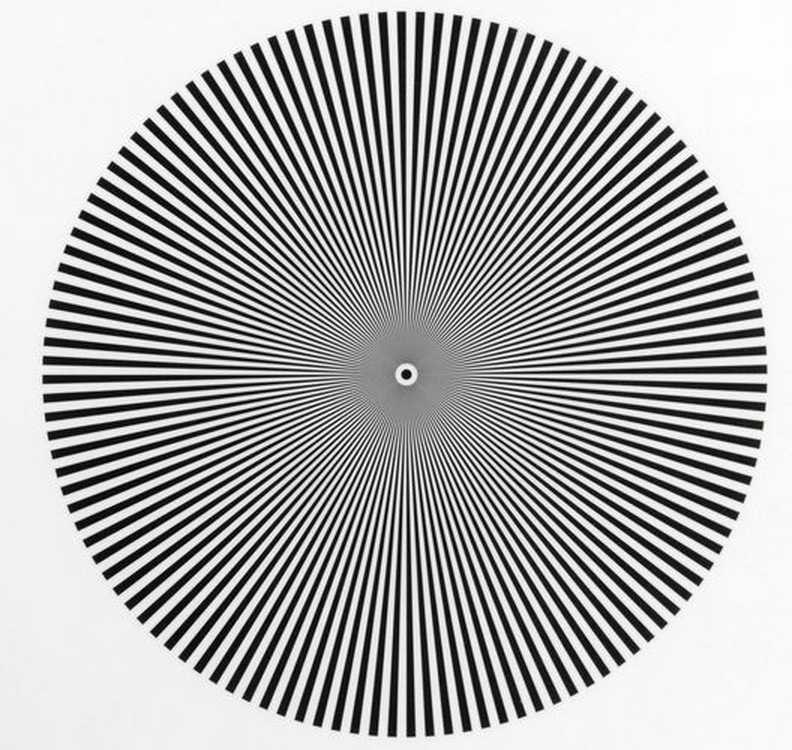
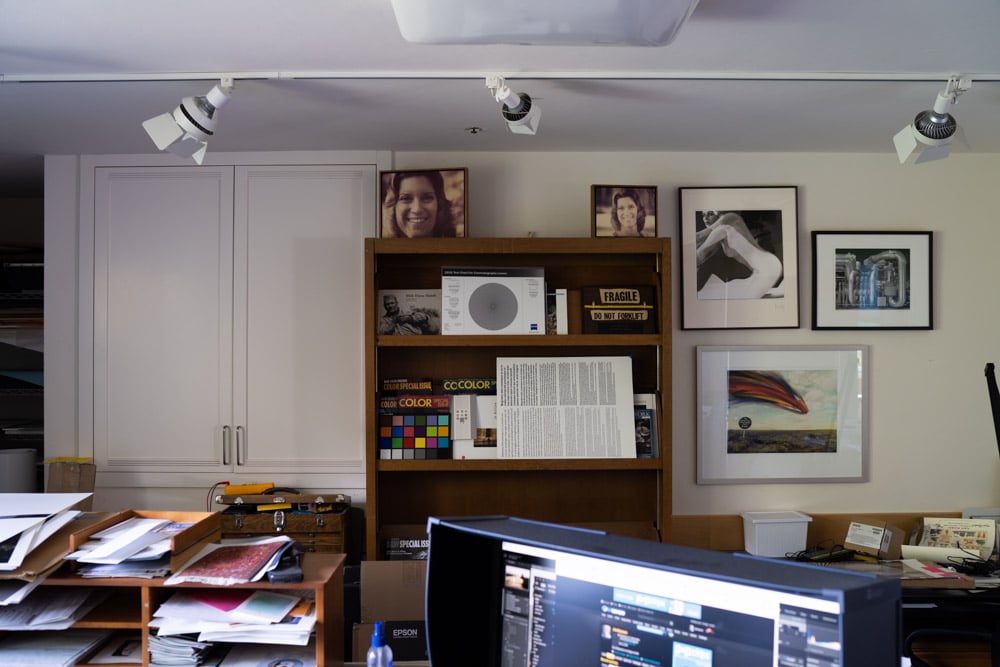
SXM is utilized in material science, life science, cultural heritage, environmental science, nano-technology and archaeology (Mino et al., 2018 ▸ Hémonnot & Köster, 2017 ▸ Cotte et al., 2018 ▸). Scanning hard X-ray microscopy (SXM) offers methods for structure, morphology and composition studies of heterogeneous sample systems.
